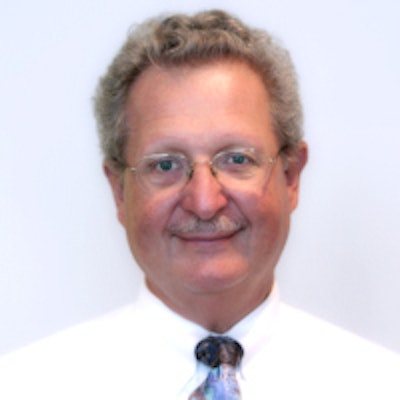
AuntMinnie.com presents the 22nd in a series of columns on the practice of ultrasound from Dr. Jason Birnholz, one of the pioneers of the modality.
I have never thought that ultrasound physics content for medical users has anything to do with either ultrasound or physics. As I see it, being rootless scientifically has limited the technical progress of our field, even while its clinical utilization and popularity have increased. It is as if we are all on the ultrasound supership on a medical ocean without a rudder.
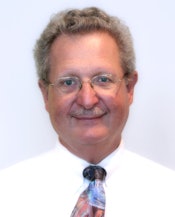
Let's take an excursion to our parent realm, x-ray imaging. I hope many of you had a chance to visit the special exhibit at the last RSNA meeting, which celebrated the centennial of the organization. There were Crookes tubes, some of the first commercial radiography equipment, and lots of anecdotes by and about the field's pioneers.
There is mystery and magic in the scenery of an invisible beam that leaves a remnant of its silent passage through the body. The earliest users, like the earliest photographers, did not have much of a clue of what they were working with, obviously. They accomplished something magnificent, but real progress started when the physical foundations for x-ray work and the clinical goals of its users were integrated.
Photons
X-rays are streams of photons, sufficiently energetic that they can cause biologic harm. There were the sad lessons of early users with repeated exposures that taught us respect and operational restraint and also led to ways to intensify and channel those destructive effects as a surgical alternative or adjunct. Magic and mystery and danger: a captivating triad.
A photon is an energy packet. Visible light are streams of photons, and when you look up at a night sky, you have to be awed by the notion of these vanishingly tiny energy parcels, traversing interstellar and even intergalactic space, eternally, at a constant velocity.
At the end of the 19th century, Planck was working on a phenomenon called black-body radiation when he had an astounding insight: that energy is conveyed by individual packets. Extant data could all be modeled by each packet having a single, discrete energy. Considering each packet as a harmonic wave, he proposed that its energy was determined solely by its frequency and that each packet had just one discrete energy value. This was expressed by E = hν, where h is a constant and ν (Greek "nu") is frequency.
Planck's constant has a value of 6.62606957 × 10-34 m2 kg/s. Perhaps the most impressive aspect of Planck's concept was that in an intellectual world where everything mathematical was always on a continuous scale with an infinite number of gradations possible between any two numerical values, nobody ever thought in terms of discrete, fixed values for heat, light, or any form of energy. The former world was a place where everything was a little blurred at some level of magnification.
That is why his concept was so outside the box or beyond the silo (if the box metaphor seems clichéd). And if you wanted to test it experimentally, you would seem to need an impossible 34-place measurement precision. It's not a surprise that virtually all of the people who worried about such matters gave the notion a double thumbs down.
Around the same time, Einstein was working on a recently described phenomenon of generating an electric current by shining a light on a piece of metal. In one direction, you have a solar cell, and in the other, liquid crystal displays. Of course, no one could have known that then. However, his observations were definitely worth a Nobel Prize, because he found that generating current depended on the frequency (i.e., color) of light, not its intensity.
In the red-orange side of the spectrum, nothing happened, but a threshold was passed, and electrons were kicked out of their stable atomic locations at the higher frequency violet end of the visible light spectrum. This demonstrated the validity of the Planck supposition and jump-started the quantum era. Einstein also derived the Planck equation by an independent means. There was some later stuff about gravity and relativity too. He has always been my hero.
When you were thinking of light photons streaming across the galaxy, did you picture them as bullets? One of the problems of any technical field is that they borrow everyday words and then have to redefine them, and they always continue to suffer the weight of semantic overtones. Patients may complain of a medical vocabulary when it is not translated into common terms, but the words we use professionally convey a distinct meaning -- except when we want them to be ambiguous.
Early quantum physicists had a problem right at the start, because they could not resolve the issue of whether photons were waves or solid particles. For the most part, they were trying to explain experimental observations and resolve an irreconcilable linguistic difference at the same time. Compounding the problem rather than illuminating it were the immutable conservation laws: Energy is neither created nor destroyed; ditto for matter, charge, and momentum. (There is spin, too, but we're not going there.)
E=MC2, right? E is energy, M is mass, and C is the velocity of a photon in a vacuum, which is a constant. One way to think of a photon is that E/M is a constant and that what happens is a probabilistic, dynamic equilibrium shifting from mostly wave-like E to the mostly particulate M part that conveys momentum. When the photon at a high enough frequency encounters an electron, there will be an elastic collision. This is Compton scattering (1924), which is the atom-level interaction we use for x-ray imaging.
As particles, photons carry no charge. There is no antiphoton. As waves, photons with the same frequency can occupy the same space and time. Particles that have this property are referred to as "bosons." Photons consist of a stable pairing of a quark and antiquark; "waves" are something like a shimmering probabilistic field, instead of what we think of when we are watching the surface of an ocean.
A photon is the messenger of electromagnetism, one of the four basic forces that shape our universe. One of the ways of regarding photons is as an information tidbit. Seen this way, a photon coming within the field of an electron or proton conveys an instruction of what the larger object has to do. Keep this concept somewhere in the back of your mind for now.
6.0221413 × 1023
I assume you all recognize Avogadro's constant, which is the number of atoms in a mole of any substance. This was identified way back in 1811, a pretty humbling example of what simple observations and brain power can achieve. It's a mighty big number. One mole of water weighs 18.6 grams, a tad more than half an ounce. I remember hearing that there are more water molecules in a glass of water than there are glasses of water in all our oceans. Another example is that a mole stack of 1-inch donuts would extend from your morning coffee shop to a place about 1.6 million light years away, well beyond the Milky Way.
I'm mentioning this because we never deal with individual photons in our work or daily lives, but instead with mind-boggling numbers of them at any one time. A 100-watt bulb spouts out some 1020 photons every second. There are perhaps 1014 cells in the body, and each of those has scads of organelles, molecules, and atoms. It's easy to lose sight of the truly vast numbers of participants in an x-ray exposure, radionuclide scan, or one of our ultrasound imaging sessions.
Phonons
Like our x-ray-wielding cousins, we in ultrasound deal with photons too! E = hν applies, as always. The energy packets that we use comprise the phonon part of the spectrum, and they have some special properties.
Photons of light, radio, and x-rays are identified as electromagnetic and are described as having out-of-phase harmonic electrical and magnetic oscillations. These all propagate through a vacuum at the speed of light, independent of frequency. Propagation velocity is less in solid media, proportional to its index of refraction: about two-thirds the speed of light in glass and about half C in diamond.
Light spread by a prism maps the energy level as frequency increases with each step of red, orange, yellow, green, blue, indigo, and violet (ROYGBIV). For oscillating waves, like all electromagnetic ones, one can characterize them on the basis of frequency, cycles per unit time, or wavelength, which is the length traversed by one cycle of a propagation wave in a medium. So f (instead of nu, to avoid confusion with v for velocity) = v/λ. Lambda is the symbol for wavelength, a value that is typically used for image-related work since you cannot resolve structures smaller than a wavelength. The wavelength for light is in the range of 5 × 10-7 m and for a 70-KeV x-ray photon, it is about 10-11 m; the size of a water molecule is 3 × 10-10 m.
Ultrasonic phonons are orders of magnitude less energetic than light and x-ray photons. They cannot propagate through a vacuum or gas. Atoms in a tight lattice of a crystal and molecules in a liquid are each vibrating in accord with their characteristic energy level. Phonons propagate as vibrational energy. There is a limiting frequency for vibration in solids that is about 1013 Hz, which is in the infrared domain and the point at which there is potential ionization in tissues, plainly not an issue for our weak but valiant lower frequency phonons.
Like their higher-energy cousins, phonons have an orthogonal, out-of-phase wave structure. Because they do not change the center of mass of oscillating atoms as they propagate, phonons do not have mass, are fully wave-like, and they are unaffected by gravity.
When a pure wave is dissipated, it is gone forever. Phonons can be created from more energetic photons after energy-depleting interactions. Under some conditions, phonons may cause inelastic diffraction of light photons, known as the Brillouin effect. There are some well-founded speculations that vibrational phonon interactions are an integral step in the energy transfer mechanism of photosynthesis.
Phonons are low-energy photons with a distinct identity, worthy of their own name. They can also be thought of as messengers of vibrational energy operating in a thermodynamic space rather than an electrodynamic domain, which includes chemical bonding for the molecules in the phonon landscape.
Who among us might not appreciate the cuteness or the grim determination of a single energy phonon gliding like heat through the molecular soup of some part of the body at just 0.00051% of the speed of an x-ray photon. It causes no damage. In its passage, it is jostled and bounced around over the mosh pit of a vast number of perpetually vibrating water molecules. Each water molecule has strong 104° oxygen hydrogen bonds within itself and two hydrogen bonds to adjacent water molecules. Two of the bonds are short and two are long.
The phonon contributes to this bond-length dance and is reinforced by it. At some point it smacks up against a wall bigger than its wavelength and is sent off on another rave along a new trajectory. Substitute triple-stranded superhelices of collagen for the generic "wall" and you have the essence of what information is conveyed to us by our dogged traveler.
The next step
Ultrasound has always struggled academically and intellectually, because we have collectively not understood that our foundations have a basic commonality with the older and more familiar, higher energy, photon-based forms of body imaging. This has a lot to do with status of the method, when it is selected for use, and our pride in using it.
We urge our patients to become better informed and interact with their care providers, including those times when an imaging study is on the diagnostic menu. The global, medical ultrasound community has not been as diligent in educating itself about the intricacies of what we do. Can we continue to rely on stolid-state physics?
I used to ask audiences about where ultrasound comes from, expecting sonar as the common answer. The answer I would have liked to have heard, even once, was the physical exam technique of percussion. As you know, this was used initially on wine vats and then on chests. Rapping briskly with a finger and either feeling the vibrations or listening for the sound is no different from smashing a quartz crystal underwater and listening or otherwise sensing acoustic reverberations from an iceberg or submarine. We do things a lot better now than when I made my own first ultrasound images in 1968, but we pretty much all do it the same way now that we did then.
In the real world, we deal with aggregated beams of gazillions of phonons traversing a medium that is far from ideal. Put another way, lack of coherence of the acoustic signals for interrogating tissues makes for a whole lot of noise in the images we have to work with. Think about this and do a search for the term "SASER." That is an example of how integrating our phononic foundations will shape our future. Phonons are ultralovely heirs of their magical, mysterious, and dangerous relatives whose potential is yet to have been exploited fully.
Dr. Jason Birnholz was one of the few advanced academic fellows of the James Picker Foundation, and he has been a professor of radiology and obstetrics. He is a fellow of the American College of Radiology and the Royal College of Radiology, and he was an associate fellow of the American College of Obstetricians and Gynecologists.
The comments and observations expressed herein do not necessarily reflect the opinions of AuntMinnie.com.